Monday, February 14, 2011
REDOX
Redox (shorthand for oxidation-reduction) reactions describe all chemical reactions in which atoms have their oxidation number (oxidation state) changed. This can be either a simple redox process, such as the oxidation of carbon to yield carbon dioxide (CO2) or the reduction of carbon by hydrogen to yield methane (CH4), or a complex process such as the oxidation of sugar (C6H12O6) in the human body through a series of complex electron transfer processes.
The term comes from the two concepts of reduction and oxidation. It can be explained in simple terms:
- Oxidation is the loss of electrons or an increase in oxidation state by a molecule, atom, or ion.
- Reduction is the gain of electrons or a decrease in oxidation state by a molecule, atom, or ion.
Though sufficient for many purposes, these descriptions are not precisely correct. Oxidation and reduction properly refer to a change in oxidation number — the actual transfer of electrons may never occur. Thus, oxidation is better defined as an increase in oxidation number, and reduction as a decrease in oxidation number. In practice, the transfer of electrons will always cause a change in oxidation number, but there are many reactions that are classed as "redox" even though no electron transfer occurs (such as those involving covalent bonds).
Non-redox reactions, which do not involve changes in formal charge, are known as metathesis reactions.
|
Oxidizing and reducing agents
In redox processes the reductant transfers electrons to the oxidant. Thus, in the reaction, the reductant or reducing agent loses electrons and is oxidized, and the oxidant or oxidizing agent gains electrons and is reduced. The pair of an oxidizing and reducing agent that are involved in a particular reaction is called a redox pair.
Oxidizers
Substances that have the ability to oxidize other substances are said to be oxidative or oxidizing and are known as oxidizing agents, oxidants, or oxidizers. Put another way, the oxidant removes electrons from another substance, and is thus itself reduced. And, because it "accepts" electrons, it is also called an electron acceptor.
Oxidants are usually chemical elements or substances with elements in high oxidation numbers (e.g., H2O2, MnO−
4, CrO3, Cr2O2−
7, OsO4) or highly electronegative substances/elements that can gain one or two extra electrons by oxidizing an element or substance (O, F, Cl, Br).
Reducers
Substances that have the ability to reduce other substances are said to be reductive or reducing and are known as reducing agents, reductants, or reducers. That is, the reductant transfers electrons to another substance, and is thus itself oxidized. And, because it "donates" electrons, it is also called an electron donor. Electron donors can also form charge transfer complexes with electron acceptors.
Reductants in chemistry are very diverse. Electropositive elemental metals, such as lithium, sodium, magnesium, iron, zinc, aluminium, carbon, are good reducing agents. These metals donate or give away electrons readily. Hydride transfer reagents, such as NaBH4 and LiAlH4, are widely used in organic chemistry,[1][2] primarily in the reduction of carbonyl compounds to alcohols. Another method of reduction involves the use of hydrogen gas (H2) with a palladium, platinum, or nickel catalyst. These catalytic reductions are used primarily in the reduction of carbon-carbon double or triple bonds.
Examples of redox reactions
A good example is the reaction between hydrogen and fluorine in which hydrogen is being oxidized and fluorine is being reduced:
- H2 + F2 → 2 HF
We can write this overall reaction as two half-reactions:
the oxidation reaction:
- H2 → 2 H+ + 2 e−
and the reduction reaction:
- F2 + 2 e−
→ 2 F−
Analyzing each half-reaction in isolation can often make the overall chemical process clearer. Because there is no net change in charge during a redox reaction, the number of electrons in excess in the oxidation reaction must equal the number consumed by the reduction reaction (as shown above).
Elements, even in molecular form, always have an oxidation number of zero. In the first half-reaction, hydrogen is oxidized from an oxidation number of zero to an oxidation number of +1. In the second half-reaction, fluorine is reduced from an oxidation number of zero to an oxidation number of −1.
When adding the reactions together the electrons cancel:
-
H2 → 2 H+ + 2 e− F2 + 2 e− → 2 F− H2 + F2 → 2 H+ + 2 F−
And the ions combine to form hydrogen fluoride:
- H2 + F2 → 2 H+ + 2 F− → 2 HF
Displacement reactions
Redox occurs in single displacement reactions or substitution reactions. The redox component of these types of reactions is the change of oxidation state (charge) on certain atoms, not the actual exchange of atoms in the compounds.
For example, in the reaction between iron and copper(II) sulfate solution:
- Fe + CuSO4 → FeSO4 + Cu
The ionic equation for this reaction is:
- Fe + Cu2+ → Fe2+ + Cu
As two half-equations, it is seen that the iron is oxidized:
- Fe → Fe2+ + 2 e−
And the copper is reduced:
- Cu2+ + 2 e−
→ Cu
Other examples
- The oxidation of iron(II) to iron(III) by hydrogen peroxide in the presence of an acid:
-
- Fe2+ → Fe3+ + e−
- H2O2 + 2 e− → 2 OH−
- Overall equation:
-
- 2 Fe2+ + H2O2 + 2 H+ → 2 Fe3+ + 2 H2O
- The reduction of nitrate to nitrogen in the presence of an acid (denitrification):
-
- 2 NO3− + 10 e− + 12 H+ → N2 + 6 H2O
- Oxidation of elemental iron to iron(III) oxide by oxygen (commonly known as rusting, which is similar to tarnishing):
-
- 4 Fe + 3 O2 → 2 Fe2O3
- The combustion of hydrocarbons, such as in an internal combustion engine, which produces water, carbon dioxide, some partially oxidized forms such as carbon monoxide, and heat energy. Complete oxidation of materials containing carbon produces carbon dioxide.
- In organic chemistry, the stepwise oxidation of a hydrocarbon by oxygen produces water and, successively, an alcohol, an aldehyde or a ketone, a carboxylic acid, and then a peroxide.
Balancing redox reactions
Describing the overall electrochemical reaction for a redox process requires a balancing of the component half-reactions for oxidation and reduction. For reactions in aqueous solution, this generally involves adding H+, OH−, H2O, and electrons to compensate for the oxidation changes.
Acidic media
In acidic media, H+ ions and water are added to half reactions to balance the overall reaction.
For example, when manganese(II) reacts with sodium bismuthate:
-
Unbalanced reaction: Mn2+(aq) + NaBiO3(s) → Bi3+(aq) + MnO4− (aq) Oxidation: 4 H2O(l) + Mn2+(aq) → MnO−
4(aq) + 8 H+(aq) + 5 e−Reduction: 2 e−
+ 6 H+ + BiO−
3(s) → Bi3+(aq) + 3 H2O(l)
The reaction is balanced by scaling the two half-cell reactions to involve the same number of electrons (multiplying the oxidation reaction by the number of electrons in the reduction step and vice versa):
- 8 H2O(l) + 2 Mn2+(aq) → 2 MnO−
4(aq) + 16 H+(aq) + 10 e− - 10 e−
+ 30 H+ + 5 BiO−
3(s) → 5 Bi3+(aq) + 15 H2O(l)
Adding these two reactions eliminates the electrons terms and yields the balanced reaction:
- 14 H+(aq) + 2 Mn2+(aq) + 5 NaBiO3(s) → 7 H2O(l) + 2 MnO−
4(aq) + 5 Bi3+(aq) + 5 Na+(aq)
Basic media
In basic media, OH− ions and water are added to half reactions to balance the overall reaction.
For example, in the reaction between potassium permanganate and sodium sulfite:
-
Unbalanced reaction: KMnO4 + Na2SO3 + H2O → MnO2 + Na2SO4 + KOH Reduction: 3 e−
+ 2 H2O + MnO4− → MnO2 + 4 OH−Oxidation: 2 OH− + SO32− → SO42− + H2O + 2 e−
Balancing the number of electrons in the two half-cell reactions gives:
- 6 e−
+ 4 H2O + 2 MnO4− → 2 MnO2 + 8 OH− - 6 OH− + 3 SO32− → 3 SO42− + 3 H2O + 6 e−
Adding these two half-cell reactions together gives the balanced equation:
- 2 KMnO4 + 3 Na2SO3 + H2O → 2 MnO2 + 3 Na2SO4 + 2 KOH
PRE-U EXPERIMENT
MAKING AND TESTING A SIMPLE GALVANIC CELL
PURPOSE
The purpose of this experiment is to introduce the student to electrochemistry and oxidation-reduction reactions through the construction and operation of a simple galvanic cell.
DESCRIPTION
This experiment is appropriate for a general or first-year college-prep course. An inexpensive cell is made from materials obtained locally. Students test the cell by connecting it to a 1.5-volt flashlight lamp and observing whether or not the lamp will light. In addition, four or more of these cells can be connected in series to form a battery that can power a small radio or other device that operates on direct current.
TIME REQUIRED
Two to three labs periods.
MATERIALS
- Chemicals
- 0.5 M copper(II) sulfate (dissolve 125 g CuSO4·5H2O in distilled or deionized water and dilute to 1.00 liter)*
- 0.5 M sodium sulfate (dissolve 71 grams of Na2SO4 in distilled or deionized water and dilute to 1.00 liter)
- zinc, copper, aluminum, and magnesium strips (approximately 1 cm x 10 cm)*
- Equipment
- 250-mL beaker*
- dialysis tubing*
- hook-up wire or bell wire
- 1.5-volt flashlight lamp with less than 100 milliamp rating
- alligator clamps
- crimping tool
- soldering iron and resin core solder
- clamps(for dialysis tubing)
- sandpaper or steel wool
HAZARDS
Avoid contact with solutions. Goggles must be worn throughout experiment.
MODIFICATIONS/SUBSTITUTIONS
- Copper sulfate pentahydrate is available as root killer at garden supply stores.
- Aluminum, from aluminum cans, may be used if it is sanded well on both sides. Aluminum gutter nails may also be used.
- Copper tubing or fittings may be used in place of copper strips.
- Zinc can be obtained from old dry cell battery casings. This should be done carefully to avoid contact with caustic chemicals in battery.
- Sausage casings can be used in place of dialysis tubing but diffusion is extremely rapid. Dialysis tubing is readily available in biology labs.
- Baby food jars or other open glass jars may be used instead of beakers.
PROCEDURE
- Prepare a test lamp by soldering wire test leads to a 1.5-volt flashlight lamp. Attach alligator clips to the ends of the leads.
- Obtain strips of magnesium and copper, each strip should be 2.5 cm longer than the height of the beaker being used. Sand the strips until they are shiny.
- Tie a knot with string or use a non-reactive clamp in one end of dialysis tubing that has been soaked in distilled water. The length of tubing should be long enough so that it overlaps the edge of the beaker by 2.5 cm.
- Fill the dialysis tubing with the prepared copper(II) sulfate solution. Place the copper strip in this piece of dialysis tubing that is now filled with copper(II) sulfate solution and use string or a rubber band to secure the top of the dialysis tubing around the copper. Leave 2.5 cm of copper sticking out of the tubing.
- Fill the beaker with the prepared sodium sulfate solution, place both the dialysis tubing, containing the copper and copper(II) sulfate solution, and the magnesium strip in the beaker. Place the magnesium strip as far away as possible from the dialysis tubing. Secure both the magnesium strip and the copper strip in the dialysis tubing in the beaker by bending them over the edge of the beaker.
- Complete the circuit by attaching one alligator clip to the copper strip and the other to the magnesium strip.
- Observe and record any activity taking place at the metal strips, the solution (especially color changes), and the test lamp.
- Connect several cells in series and operate a small radio or some other direct current device.
- Try making cells with zinc or aluminum in place of the magnesium.
DISPOSAL
All solutions may be flushed down the drain with water.
DISCUSSION
The following discussion applies when copper and magnesium strips are used as the metal strips. If other metals are used, similar explanations can be used. Mg(s) + Cu2+(aq)This reaction is an oxidation-reduction reaction in which the magnesium is oxidized by the copper to Mg2+ ions. The result of this reaction is a transfer of electrons which provides the power required to light the flashlight lamp." src="file:///F:/galvanic%20experiment_files/rightarrow.htm" align="BOTTOM" border="0" hspace="4"> Mg2+(aq) + Cu(s) + 1.5v
Students performing this experiment should be familiar with the following terms: anode, cathode, oxidizing agent, reducing agent, and electromotive series. Students should be presently studying a unit on electrochemistry and oxidation-reduction reactions in order to fully understand the "chemistry" of this experiment.
TIPS
- The metal strips used in this experiment should have a large surface area to minimize resistance within the cell.
- Use a voltmeter instead of the light bulb to eliminate the need for soldering.
- Aluminum strips may not work unless sanded thoroughly and acid treated with 6.0 M HCl.
- Soldering techniques should be mastered by teacher so that any soldering can be
REFERENCE
Summerlin, L.R., and Ealy, J.L. Jr, Chemical Demonstrations-A Sourcebook for Teachers, American Chemical Society, Washington D.C, 1985, p. 115. This experiment is adapted from this source.
ELECTROCHEMICAL REACTION
Here, each of the reactant is taken in a separate container in contact with a rod/sheet of a metallic-conductor (electronic conductor) called an electrode. Electrical contact between the two reactants is established by placing a conducting salt bridge in-between.
Fig: 9.2 - Experimental set up for an indirect redox reaction
The species capable of losing electrons transfers them to the electrode placed in it. This makes the metallic conductor or electrode negatively charged due to the accumulation of electrons. On the other hand, the species capable of gaining electrons accepts them from the metallic conductor (electrode) placed in it. As a result this metallic conductor electrode) gets positively charged due to the deficiency of electrons. When a metallic wire connects the two electrodes, the electrons flow from the negatively charged electrode to the positively charged electrode. Thus, in an indirect redox reaction, electrons flow in a particular direction through an external conducting wire connecting the two electrodes.
This flow of electrons generates electricity, which can be used for doing some useful work.
Thus, in an indirect redox reaction, the decrease in the chemical energy is liberated in the form of electrical energy.
An electrochemical reaction differs from a chemical reaction in the following respects.
Chemical reaction | Electrochemical reaction |
Electron transfer from one species to another takes place directly in the same medium. | Electron transfer from one species to another takes place indirectly through electrodes. |
Energy is liberated in the form of heat, light and sound. | Energy is liberated in the form electrical energy. |
The chemical reaction is instantaneous proceeding at a finite rate. | This reaction takes place only on the application of electricity. |
Redox reactions take place in the same medium. | Redox reaction takes place separately at the Anode and cathode surface. |
Electrochemical cell
Spontaneous redox reactions are the metal displacement reactions. Therefore such reactions have been used for producing electricity. This is done by carrying out these reactions in specially designed units called electrochemical cells.
An electrochemical cell is a device used to convert chemical energy of an indirect redox reaction into electrical energy. This is also called Voltaic cell or a Galvanic cell. It is set up by dipping two electrodes (conducting rods) into the same or two different electrolytes. No reaction takes place inside the cell until a conducting wire joins the two electrodes.A typical metal displacement reaction is the reaction between zinc metal and copper sulphate solution i.e.,
The electrochemical cell based on this reaction is set up as follows.
Zinc sulphate solution is taken in a beaker and a zinc rod is dipped in to it. Similarly copper sulphate solution is taken in another beaker and a copper strip is dipped in to it. An inverted U tube containing concentrated solutions of inert electrolytes such as KCl, KNO3 etc., connects the two solutions. The two openings of the U tube are plugged with porous materials like glass wool or cotton. This U tube is called as salt bridge as it acts like a bridge connecting the solutions of the two beakers. In place of salt bridge, one can also use either a paper strip, unglazed porcelain or clay porous pot or asbestos fibre for developing electrical contact between the two half-cells. When a key is inserted to complete the outer circuit, the following observations are made.- There is a flow of electrical current through the external circuit.
- The zinc rod loses weight, while the copper rod acquires weight.
- The concentration of ZnSO4 solution increases, while that of CuSO4 solution decreases.
- The two solutions in the beakers have electrical neutrality.
The above indications clearly show the overall reaction to be:

Zinc is oxidized to Zn2+ ions and go into the solution during the reaction.

The electrons released at the electrode move towards the other electrode through the outer circuit. These electrons are accepted by Cu2+ ions of CuSO4 solution. Thus the Cu2+ ions are reduced to metallic copper, which get deposited on the copper electrode.

With the onset of the chemical reaction, the zinc plate begins to dissolve and loses weight, electrons get generated and move and finally, the copper plate gains weight. Therefore, this indirect redox reaction is accompanied by the liberation of energy in terms of electric charge, which is the electrical energy. In this way the chemical reaction leads to the production of electrical energy that further helps in doing useful work (deposition of copper metal).
Fig: 9.4 - Electrochemical cell based on redox reaction of zinc and copper sulphate
Salt Bridge and Its Function
A salt bridge is a low resistance device, which establishes an electrical contact between two electrolytes not in direct contact. This is used to overcome the direct liquid-liquid junction that leads to intermixing. The salt bridge consists of a glass U-tube filled with KCl containing Agar-Agar paste, which sets into a gel. The choice of the electrolyte added to the gel depends upon the nature of the electrolytes used in the cell. However these electrolytes are inert for they should not react chemically or undergo any electrochemical reactions with the electrolytes electrically connected with them. Commonly used salts are, KCl, NH4NO3, KNO3, or K2SO4. The KCl-bridge cannot be used when any salt of lead, or silver is used in the cell because lead chloride and silver chloride are insoluble in water.
Functions of a Salt Bridge
- When in a galvanic cell two solutions are kept in separate containers, an electrical contact between the two is needed to complete the circuit. A salt bridge completes the circuit by allowing the migration of anions from one container into the salt bridge and from the salt bridge into the other container.
- The salt bridge prevents the physical transference/diffusion of the electrolytes from one container to the other.
- A salt bridge helps in maintaining the charge balance in the reactions taking place at the two containers by releasing counter ions into the solution. Other wise due to the accumulation of the respective charges in the two containers there will be no flow of electrons and the cell will stop functioning.
- A direct liquid-liquid junction is thermodynamically unstable state. The unequal rates of migration of the cations and anions across a liquid-liquid junction give rise to a potential difference across the junction. This potential difference across the liquid-liquid junction is called liquid junction potential. A salt bridge eliminates a direct contact between the two solutions, and thus minimizes the liquid junction potential.
GALVANIC CELL
A Galvanic cell, or Voltaic cell, named after Luigi Galvani, or Alessandro Volta respectively, is an electrochemical cell that derives electrical energy from chemical reactions taking place within the cell. It generally consists of two different metals connected by a salt bridge, or individual half-cells separated by a porous membrane.
Volta was the inventor of the voltaic pile, the first electrical battery. In common usage, the word "battery" has come to include a single Galvanic cell, but a battery properly consists of multiple cells.[1][2]
History
In 1780, Luigi Galvani discovered that when two different metals (copper and zinc for example) were connected together and then both touched to different parts of a nerve of a frog leg at the same time, they made the leg contract.[3] He called this "animal electricity". The voltaic pile invented by Alessandro Volta in the 1800s is similar to the galvanic cell. These discoveries paved the way for electrical batteries.
|
Description
A Galvanic cell consists of two half-cells. In its simplest form, each half-cell consists of a metal and a solution of a salt of the metal. The salt solution contains a cation of the metal and an anion to balance the charge on the cation. In essence the half-cell contains the metal in two oxidation states and the chemical reaction in the half-cell is an oxidation-reduction (redox) reaction, written symbolically in reduction direction as
- Mn+ (oxidized species) + n e−
M (reduced species)
In a galvanic cell one metal is able to reduce the cation of the other and, conversely, the other cation can oxidize the first metal. The two half-cells must be physically separated so that the solutions do not mix together. A salt bridge or porous plate is used to separate the two solutions yet keep the respective charges of the solutions from separating, which would stop the chemical reactions.
The number of electrons transferred in both directions must be the same, so the two half-cells are combined to give the whole-cell electrochemical reaction. For two metals A and B:
- An+ + n e−
A
- Bm+ + m e−
B
- m A + n Bm+
n B + m An+
This is not the whole story as anions must also be transferred from one half-cell to the other. When a metal in one half-cell is oxidized, anions must be transferred into that half-cell to balance the electrical charge of the cation produced. The anions are released from the other half-cell where a cation is reduced to the metallic state. Thus, the salt bridge or porous membrane serves both to keep the solutions apart and to allow the flow of anions in the direction opposite to the flow of electrons in the wire connecting the electrodes.
The voltage of the Galvanic cell is the sum of the voltages of the two half-cells. It is measured by connecting a voltmeter to the two electrodes. The voltmeter has very high resistance, so the current flow is effectively negligible. When a device such as an electric motor is attached to the electrodes, a current flows and redox reactions occur in both half-cells. This will continue until the concentration of the cations that are being reduced goes to zero.
For the Daniell cell, depicted in the figure, the two metals are zinc and copper and the two salts are sulfates of the respective metal. Zinc is the oxidized metal so when a device is connected to the electrodes, the electrochemical reaction is
- Zn + Cu2+ → Zn2+ + Cu
The zinc electrode is dissolved and copper is deposited on the copper electrode (as copper ions become reduced to copper metal). By definition, the cathode is the electrode where reduction (gain of electrons) takes place, so the copper electrode is the cathode. The cathode attracts cations, so has a negative charge when current is discharging. In this case, copper is the cathode and zinc the anode.
Galvanic cells are typically used as a source of electrical power. By their nature they produce direct current. For example, a lead-acid battery contains a number of galvanic cells. The two electrodes are effectively lead and lead oxide.
The Weston cell was adopted as an International Standard for voltage in 1911. The anode is a cadmium mercury amalgam, the cathode is made of pure mercury, the electrolyte is a (saturated) solution of cadmium sulfate and the depolarizer is a paste of mercurous sulfate. When the electrolyte solution is saturated the voltage of the cell is very reproducible, hence its use as a standard.
Cell voltage
The standard electrical potential of a cell can be determined by use of a standard potential table for the two half cells involved. The first step is to identify the two metals reacting in the cell. Then one looks up the standard electrode potential, E0, in volts, for each of the two half reactions. The standard potential for the cell is equal to the more positive E0 value minus the more negative E0 value.
For example, in the figure above the solutions are CuSO4 and ZnSO4. Each solution has a corresponding metal strip in it, and a salt bridge or porous disk connecting the two solutions and allowing SO42− ions to flow freely between the copper and zinc solutions. In order to calculate the standard potential one looks up copper and zinc's half reactions and finds:
- Cu2+ + 2 e−
Cu: E0 = +0.34 V
- Zn2+ + 2 e−
Zn: E0 = −0.76 V
Thus the overall reaction is:
- Cu2+ + Zn
Cu + Zn2+
The standard potential for the reaction is then +0.34 V − (−0.76 V) = 1.10 V. The polarity of the cell is determined as follows. Zinc metal is more strongly reducing than copper metal as shown by the fact that the standard (reduction) potential for zinc is more negative than that of copper. Thus, zinc metal will lose electrons to copper ions and develop a positive electrical charge. The equilibrium constant, K, for the cell is given by
where F is the Faraday constant, R is the gas constant and T is the temperature in kelvins. For the Daniell cell K is approximately equal to 1.5×1037. Thus, at equilibrium, a few electrons are transferred, enough to cause the electrodes to be charged.[4]
Actual half-cell potentials must be calculated by using the Nernst equation as the solutes are unlikely to be in their standard states,
where Q is the reaction quotient. This simplifies to
where {Mn+} is the activity of the metal ion in solution. The metal electrode is in its standard state so by definition has unit activity. In practice concentration is used in place of activity. The potential of the whole cell is obtained by combining the potentials for the two half-cells, so it depends on the concentrations of both dissolved metal ions.
The value of 2.303R/F is 0.19845×10-3 V/K, so at 25 °C (298.15 K) the half-cell potential will change by 0.05918V / n if the concentration of a metal ion is increased or decreased by a factor of 10.
These calculations are based on the assumption that all chemical reactions are in equilibrium. When a current flows in the circuit, equilibrium conditions are not achieved and the cell potential will usually be reduced by various mechanisms, such as the development of overpotentials.[5] Also, since chemical reactions occur when the cell is producing power, the electrolyte concentrations change and the cell voltage is reduced. A consequence of the temperature dependency of standard potentials is that the voltage produced by a galvanic cell is also temperature dependent.
E.M.F. OR CELL POTENTIAL OF A CELL
The electrochemical cell consists of two half cells where one of the half-cell has a higher value of reduction potential as compared to the other. As a result of this potential difference, there is a flow of electrons from the electrode with a lower reduction potential (or higher oxidation potential) to the electrode with higher reduction potential (or lower oxidation potential). The difference between the electrode potentials of the two electrodes in the electrochemical cell is known as electromotive force or cell potential of a cell. The electromotive force is commonly abbreviated as EMF (emf) and is expressed in volts. The emf of a cell may be expressed in terms of the difference in the reduction electrode potential.
EMF = Esubstance reduced - Esubstance oxidized
EMF= ER - EL or EMF= Ecathode - Eanode
where ER and EL represent electrode potential of electrode on right hand side and on the left hand side respectively. It depends upon the nature of the electrodes and the concentration of the solutions in the two half-cells.
Difference between EMF and potential difference
EMF

CELL POTENTIAL
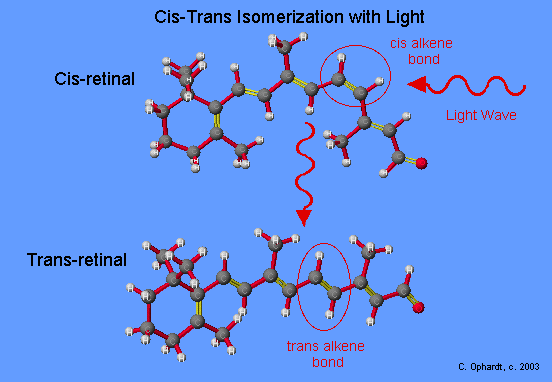
HISTORY OF ELECTROCHEMISTRY

In the mid-1780s, anatomist Luigi Galvani (Bologna, Italy) was studying the effects of atmospheric electrical discharge. One day, in his garden, he fastened brass hooks between the spinal cord of a dissected frog and an iron railing. To his amazement the frog's legs began twitching wildly, not only when lightning flashed, but also when the sky was calm. Galvani interpreted his results in terms of animal electricity (incorrect). Galvani proclaimed that the muscle retained a nerveo-electrical fluid similar to that of an electric eel. While his papers on the subject ignited research among scientists of Europe, the most significant consequence of Galvani's discovery was the concept of Galvanism which refers to the production of electrical current from the contact of two metals in a moist environment. Shortly before he died, Galvani was dismissed from his professorship at the University of Bologna, because he refused to swear allegiance to Napoleon's Republic.
Alessandro Giuseppe Volta (1745-1827)

Volta was born in Como, Italy (near Milan). In 1774, he began his first academic position as principal of the state Gymnasium in Como. In 1777, he was appointed Professor of Physics at the University of Pavia. Here he began to repeat Galvani's famous experiments with decapitated frogs. He observed that Galvani had connected brass hooks between the frog's spinal cord and an iron railing. According to Volta's interpretation, the muscle twitches were induced by current flowing between two dissimilar metals connected by the moist flesh of the frog's leg. This led him to develop the first device which demonstrated chemical production of electric current. In 1799, Volta arranged a vertical pile of metal discs (zinc with copper or silver) and separated them from each other with paperboard discs that had been soaked in saline solution. This stack became known as the voltaic pile and was the first electric battery.
In his pursuit of the current generated by his primitive batteries, Volta developed several new devices. He invented the electrophore, a forerunner of the capacitor; the condensatore, a device that detected weak electrical current; and the straw electrometer, a meteorology tool which measured atmospheric electricity. The term volt, a unit of electrical measurement, is named in his honor. In 1801, Volta was summoned to Paris to demonstrate his discovery before the Academy of Sciences. Napoleon declared his presentation a triumph, awarded him a gold medal and initiated the annual Volta Prize in his honor.
Humphry Davy (1778-1829)

At 19, Davy began studying chemistry after reading papers by Antoine Lavoisier. Within five years he was appointed Professor of Chemistry at The Royal Institution in London. In his first scientific work, Davy investigated the possible therapeutic value of inhaling various gases, using himself as a guinea pig. Since nitric oxide (laughing gas) and carbon monoxide were among the gases studied, it is surprising he survived. Davy utilizes the recently discovered voltaic pile to lay the qualitative foundations of electrochemistry. Davy isolated elemental potassium which was soon followed by sodium, barium, calcium, strontium, and magnesium. Davy later isolated boron and silicon.
Late in 1813, Davy set out on an 18 month tour of Europe accompanied by young Michael Faraday. Though neither then realized it, Davy's star was beginning to set while Faraday's was soon to rise. Faraday was to succeed Davy at The Royal Institution. Some have unkindly suggested that Davy's greatest discovery was Michael Faraday. Strictly as a chemist, Davy was the greater of the two. As a scientist, Faraday was incomparable.
Michael Faraday (1791-1867)

Although Faraday came from very humble beginnings, left school at the age of 12, and was essentially self-taught, he is acknowledged as one of the greatest of all scientists.Davy was Faraday's mentor in his early years of physics and electrochemistry research. For a time, in fact, Faraday extended and developed the research begun by Davy at the Royal Institution in London, where Faraday began his career in 1813 as Davy's Laboratory Assistant. Most of Faraday's early experiments and published papers bore the stamp of Davy's involvement.
Faraday achieved scientific prominence of his own for the First Law of Electrochemistry, developed in 1834: The chemical power of a current of electricity is in direct proportion to the absolute quantity of electricity which passes. The Second Law of Electrochemistry, also defined by Faraday, states: Electrochemical equivalents coincide, and are the same, with ordinary chemical equivalents. The work that led to these two laws also resulted in many of the modern electrochemical terms such as electrode, electrolyte, and ion.
But Faraday didn't consider himself an electrochemist; he preferred the title of natural philosopher and devoted his life to proving the interconnection of natural forces. His electrochemical research was one outcome of this effort, exploring the connection between the chemical and electrical forces of the voltaic battery.
Johann Wilhelm Ritter (1776-1810)

Ritter was born in Poland and began his career as an apothecary. He went to the University of Jena in 1796 to study science. Ritter made the first dry cell battery in 1802 and storage battery in 1803; While working with silver chloride, Ritter discovered the ultraviolet end of the spectrum. Ritter's most important contribution came in 1798 when he established an explicit connection between galvanism and chemical reactivity. He correlated the electrical effects produced by various metal couples on the muscle with differences in the metals' ease of oxidation. His suggestion that current was due to a chemical interaction between the metals was the first electrochemical explanation of this phenomenon. Fascinated by experiments in electrical excitation of muscle, Ritter performed experiments on his own body with very high voltages. Undoubtedly, these experiments led to his untimely death at the age of 33.
John Frederic Daniell (1790-1845)

Daniell was born in London and was appointed professor of chemistry at King's College, London. Daniell's research into development of constant current cells took place at the same time (late 1830s) that commercial telegraph systems began to appear. Early telegraph messages were brief and traveled short distances. Daniell's copper battery (1836) became the standard for British and American telegraph systems. In 1839, Daniell experimented on the fusion of metals with a 70-cell battery. He produced an electric arc so rich in ultraviolet rays that it resulted in an instant, artificial sunburn. These experiments caused serious injury to Daniell's eyes as well as the eyes of spectators. Ultimately, Daniell showed that the ion of the metal, rather than its oxide, carries an electric charge when a metal-salt solution is electrolyzed.